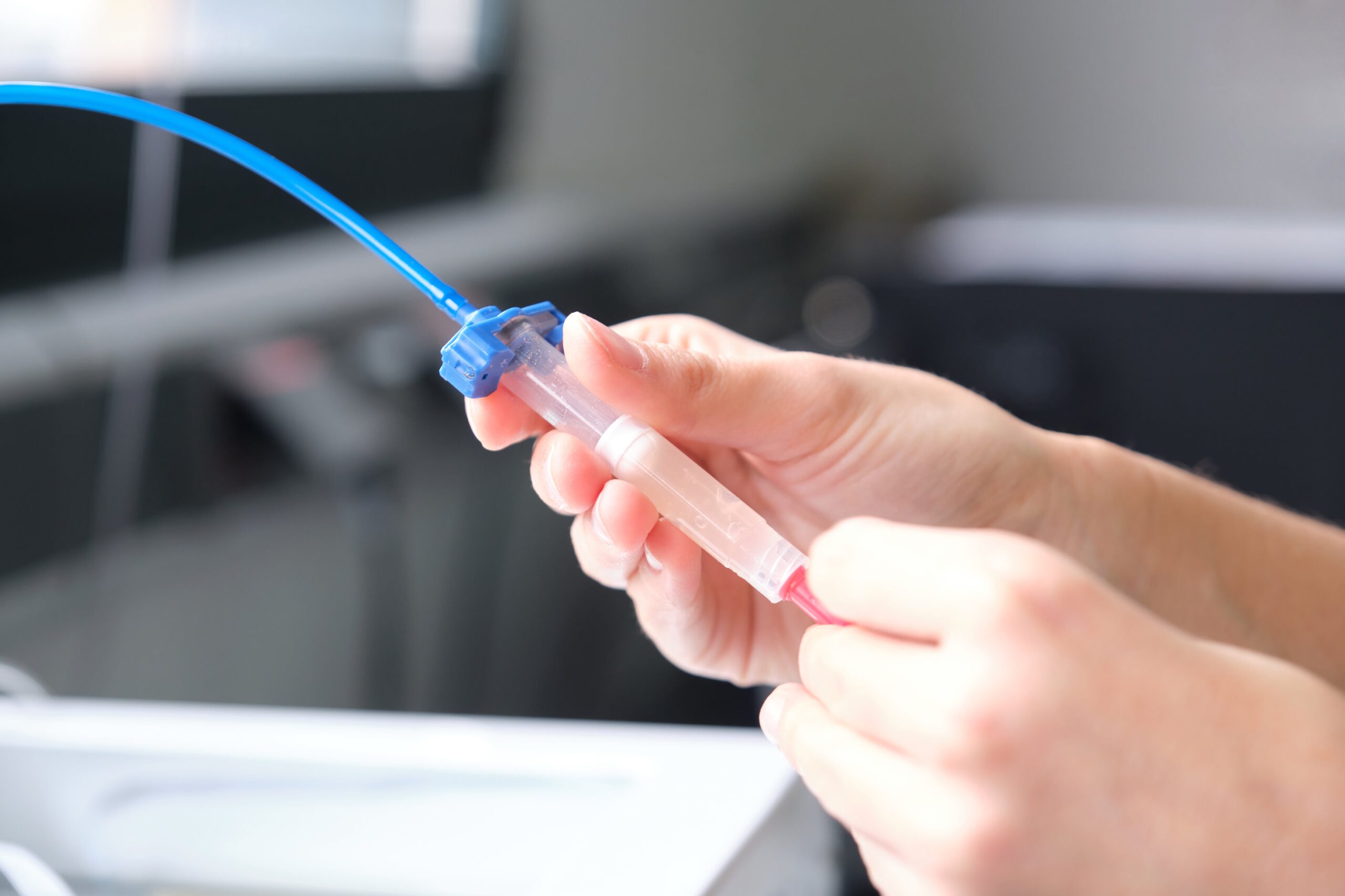
From Biomaterials to Bioinks
The transformative realm of 3D bioprinting has emerged as a beacon of hope in the landscape of regenerative medicine and tissue engineering. At the heart of this revolutionary technology lies the intricate interplay between biomaterials and living cells, orchestrated to recreate complex biological structures with unprecedented precision. Central to this intricate dance is the bioink – a composite material designed to encapsulate living cells and guide their spatial arrangement during the printing process. From synthetic polymers to natural proteins, each biomaterial brings its own set of properties and challenges to the table. As we navigate this scientific landscape, we unravel the delicate balance required to achieve printability without compromising the viability and functionality of encapsulated cells.
Bioprinting process
In 3D bioprinting, biological materials such as a patient’s own living cells, proteins, and supporting biomaterials are utilised for printing. This technology enables the fabrication of complex living tissues and organs, providing the groundwork for the production of replacement parts to replace damaged patient tissues and the creation of tissue models for research purposes. Living cells, combined with supportive biomaterials acting as structural elements, constitute what is known as bioink. The biomaterials used in bioinks can vary widely depending on their intended purpose [1]. However, each biomaterial must be biologically compatible, safe to use, and easily dispensable either as droplets or a continuous filament through the print head’s nozzle. Various printing techniques can be employed in bioprinting, with the most popular ones being inkjet or drop-on-demand bioprinting, pressure-based extrusion bioprinting, and laser-assisted bioprinting. The differences among bioprinting techniques primarily lie in how the bioink droplet or filament is formed and dispensed.
In all these different bioprinting techniques, the process begins by preparing a 3D model of the structure or form to be printed using CAD modeling software, typically reconstructed from image slices of, for example, a CT or MRI scan. The three-dimensional model is then saved in the STL file format, commonly understood by 3D bioprinters. In a separate slicing stage, the model is further segmented into two-dimensional cross-sections, which the 3D printer follows to gradually build the object by adding material layer by layer. Depending on the material and printing technique, the printed material layer either gels or solidifies immediately upon exiting the nozzle onto the printing bed or after a certain period [2]. Rapid gelation is indeed a crucial requirement for a good bioink, as, without this property, the printed structure may lose its three-dimensional shape due to the influence of the weight of the upper layers.
Thermoplastics for 3D printing
Various synthetic and natural polymers have been researched and tested for the biomaterials, or bioinks, used in 3D bioprinting. Synthetic biomaterials, such as thermoplastic polymers like polyglycolide (PGA) and polylactide (PLA), are mechanically robust materials, but their printing process often requires high temperatures or toxic solvents, making it challenging to print living cells simultaneously. Instead, cells can be seeded into pre-made porous support structures after the printing process. Synthetic thermoplastics can be employed as support structures for natural biomaterials in a technique known as hybrid bioprinting. In this approach, mechanically strong structural support is first printed from the thermoplastic, and then in the subsequent stage, cells are dispensed into the pores using a soft hydrogel. [3]
Polylactide and its stereoisomers, especially poly-L-lactide (PLLA), have been used in the fabrication of support structures and implants for applications such as microchannels and porous bone tissue engineering (Figure 1). PLA is a hydrophobic polyester that is biocompatible and slowly biodegradable. Due to its hydrophobic nature, it needs to be combined with another hydrophilic polymer to form a sufficiently water-retaining material for cells. Polyglycolide (PGA), similar to polylactide, is a hydrophobic polyester that degrades hydrolytically. PGA degrades slightly faster than PLA because it is less hydrophobic. These polymers can also be combined to form a bio-compatible poly(lactide-co-glycolide) (PLGA) copolymer, whose degradation rate can be controlled by adjusting factors such as molecular weight or the relative proportions of monomers. [4]
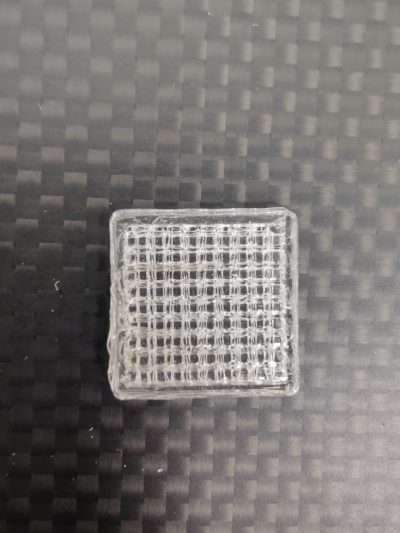
Hydrogels as bioinks
Hydrogels are the most common example of natural biomaterials, although synthetic hydrogels can also be produced. Hydrogels consist of crosslinked polymers that form a three-dimensional network structure. Their water content is very high, exceeding 99%, making them excellent soft tissue mimics. The water within the structure of hydrogels allows the diffusion of water-soluble molecules through the hydrogel matrix, a crucial aspect for replicating in vivo conditions where molecules move from the bloodstream through the extracellular matrix to the cells.
The mechanical properties of hydrogels vary somewhat depending on the type of crosslinking bonds, but in general, they can be described as viscoelastic, similar to tissues. The mechanical properties depend on the polymers used, their concentration, the nature of the bonds (covalent or non-covalent), and the degree of crosslinking. However, the mechanical strength of hydrogels is not as robust as that of thermoplastics, prompting efforts to enhance their mechanical strength by incorporating nanoparticles or inorganic components into the bioinks. In general, unmodified hydrogels are not well-suited for replacing the strongest tissues, such as bone, teeth, and cartilage. [5]
Synthetic hydrogels
Examples of synthetic hydrogels include materials based on polyethylene glycol, such as PEG acrylate (PEGDA) and polyacrylamide (PAAm)-based hydrogels. The molecular weight and distribution of synthetic hydrogel materials can be easily controlled, unlike natural hydrogels. By controlling the molecular weight, the mechanical properties, such as the elastic modulus, can be further modified to better match the characteristics of the target tissue. However, the cytocompatibility, or cell compatibility, of synthetic hydrogel materials is inferior to that of natural-based materials, which are inherently bioactive and thus provide an optimal environment for cell and tissue integration. [6]
Polyethylene glycol (PEG) is one of the most commonly used synthetic hydrogels. Although PEG does not elicit an immune response from cells, cells poorly adhere to it, requiring modifications such as the attachment of functional groups or proteins. Diacrylation is a common modification that produces light-crosslinkable PEG diacrylates (PEGDA). Polyacrylamide (PAM) is a covalently crosslinked hydrogel with good mechanical properties. The mechanical properties of polyacrylamide can be precisely tailored by modifying the length of the polymer chain. It is often used in hybrid hydrogels to impart desired mechanical properties to the structure. [6] Polyvinyl alcohol (PVA) is often used as a sacrificial material in the fabrication of hollow or porous structures due to its water solubility. After printing, the PVA-printed areas can be subsequently dissolved, creating a tubular or porous structure. The crosslinks between PVA polymers are formed by hydrogen bonds, making them mechanically less strong compared to hydrogels made from materials such as polyacrylamides. [7]
Natural hydrogels
Natural polymers can be either polysaccharides, such as alginate, chitosan, and hyaluronic acid, or polypeptides, such as gelatin, collagen, and fibrin, which are naturally found in the extracellular matrix. Although natural hydrogels exhibit excellent cell compatibility due to their various cell-adhesive ligands, their use is limited by relatively weak mechanical properties. Type I collagen is a common protein in the extracellular matrix and has been extensively used in hydrogels due to its excellent cell adhesion capabilities. Collagen contains numerous integrin-binding sites, allowing cells to attach to collagen fibers. Collagen forms a gel at room temperature and does not re-dissolve upon cooling, requiring the gelatinisation process to be performed in a cold environment during preparation and printing with a cooled print head. However, due to its weak mechanical properties, collagen lacks immediate shape retention after printing, and it is recommended to be printed using the so-called FRESH method, where collagen is printed into a supportive gelatin bath to enhance structural stability immediately after printing. [8]
Gelatin, on the other hand, is a polymer obtained from collagen through hydrolysis, retaining some cell-binding sites, such as the RGD peptide formed by arginine, glycine, and asparagine. This enhances cell adhesion. Gelatin undergoes thermoreversible gelation, initially liquefying upon heating and then solidifying into a gel upon cooling. Gelatin’s methacrylated version, GelMA, is a highly popular biomaterial for bioink due to its photosensitivity (Figure 2). By controlling the degree of photopolymerisation, it is possible to precisely regulate when and to what extent covalent crosslinks are formed in GelMA during the bioprinting process. The amount of crosslinking can further be adjusted to control the mechanical properties of the printed object, such as the stiffness of the hydrogel, a significant factor influencing cell interactions. [9]
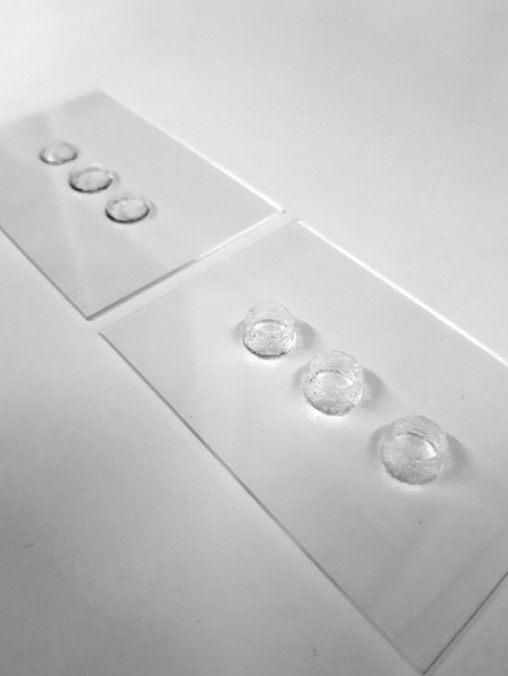
An extremely popular polysaccharide bioink is alginate, derived from brown algae, capable of forming crosslinks through ionic bonds, primarily with calcium ions. Alginate has a structure resembling the extracellular matrix, exhibiting good biocompatibility and high viscosity in gel form. Alginate, in its natural state, lacks interaction with cells; therefore, it is often modified by attaching molecules that bind to cells (such as protein sequences, e.g., RGD peptide sequence) or mixed with other cell-adhesive materials. However, the use of alginate as a bioink is hindered by challenges in controlling the gelation mechanism and regulating the balance of calcium ions, especially in more complex bioinks (Figure 3). Incorrect gelation conditions may lead to unstable prints or poor cell viability. Additionally, alginate’s high viscosity can impede printability, reduce the precision of the printed structure, and lead to clogging of the printing needle. Conversely, excessively low calcium content resulting in low viscosity can lead to structural breakdown or imprecision. [10]
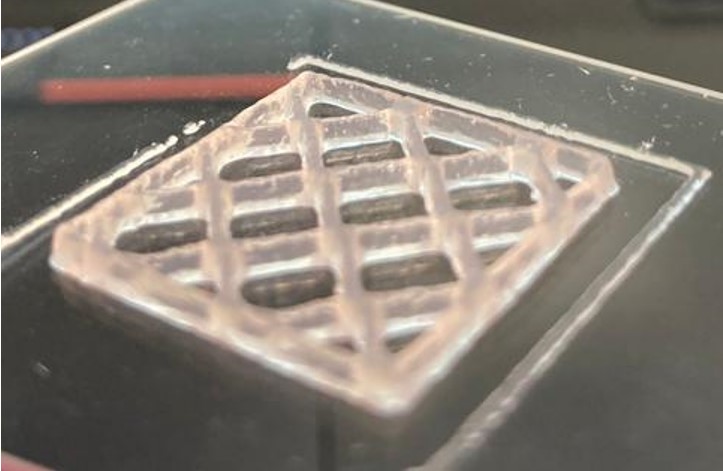
Hyaluronic acid is a common linear polysaccharide found in the extracellular matrix, particularly in human tissues such as connective tissues, synovial fluid, and eye tissues. As a natural component of the human body, hyaluronic acid is highly biocompatible, well-tolerated, and generally does not cause adverse effects or immune reactions, making it safe for use in medical applications. Hyaluronic acid is highly hydrophilic, meaning it can bind significant amounts of water. This property allows for moisture retention and the creation of a natural environment for cells. When used as a bioink, hyaluronic acid helps maintain cellular moisture, promoting cell viability and function. [11]
Despite its excellent properties, the use of hyaluronic acid as a bioink can be challenging due to variations in viscosity based on molecular weight and concentration. Controlling the viscosity of the bioink is crucial for printability and the precision of printed structures. Additionally, managing the gelation mechanism of hyaluronic acid can be challenging, especially in bioprinting. Gel formation often requires additives or reactions, such as crosslinking agents or light-induced curing. Optimizing the gelation process is essential to enable cell encapsulation and the formation of precise three-dimensional structures. Hyaluronic acid may be relatively expensive compared to other alternatives. Its production is complex and requires specific manufacturing methods, which may impact its economic feasibility on a large scale or in cost-sensitive applications. [11]
An ideal bioink
The printability of bioink is a crucial aspect of bioprinting. Ink viscosity, rheological properties, and processing time affect the accuracy and efficiency of printing. For 3D bioprinting, the bioink should ideally be pseudoplastic or shear-thinning. In pseudoplastic materials, internal bonds open or molecular chains orient under the influence of pressure or shear forces, such as when passing through a thin needle during printing, causing the material to become more fluid, i.e., its viscosity decreases. Once the external pressure is removed, the bioink can restore the bonds or rearrange its molecular chains, returning the viscosity to its initial level. To maintain the shape retention of the printed structure, it is crucial that these changes occur rapidly, allowing the printed structure to preserve its shape. Pseudoplasticity also prevents needle clogging and enables the use of lower printing pressure, which is important for the viability of cells within the bioink. [12]
In addition to pseudoplasticity, printing is possible if the bioink material is liquid under certain conditions but quickly gels by changing the environment. Modifiable environmental factors can include temperature and pH. However, for the shape retention of the printed structure, it is essential that the gel-liquid-gel changes occur within a narrow range of conditions and in a short period. Moreover, when printing with cells, it is crucial to ensure that the environmental conditions do not harm the cells during printing. Even nearly liquid bioinks can be printed if the material can be modified during or after printing, for example, through UV-induced crosslinking. In this case, the mechanical properties of the material are enhanced by forming covalent bonds between polymer chains. However, when using a UV-based crosslinking method, it should be noted that a high number of covalent bonds in the printed structure may make the gel too dense for cells. If the gel’s porosity decreases significantly, cells may not grow uniformly in the structure, let alone receive sufficient oxygen and nutrients. In such cases, the biocompatibility of the bioink and the viability of the embedded cells may deteriorate. Therefore, developing an optimal bioink is always a balancing act between biocompatibility and printability and shape retention. Recent research has focused on developing novel advanced bioinks that are both cell-friendly and highly printable. This goal is pursued by adjusting the physical, chemical, and biological properties of the polymers. [13]
Conclusions
Although 3D bioprinting is still in its developmental stages, it is believed to revolutionise medicine in the future, enabling the development of new treatment modalities such as tissue repair, organ replacement, and disease treatment. However, before reaching this goal, more advanced bioinks must provide a better platform for printing living cells without compromising printing accuracy.
To address the challenges in advancing bioink technologies, the utilization of a comprehensive biomaterial database becomes crucial. The BIOMATDB project, with its focus on creating an advanced Biomaterial Database, plays a pivotal role in this context. The database, which will be accessible via www.biomaterialdatabase.com in the future, provides detailed information on the chemical-physical, biological, and toxicological properties of various biomaterials. This valuable resource serves a wide variety of end-users, including researchers, companies, and clinicians, facilitating informed decision-making in the development and application of bioinks for 3D bioprinting.
In addition to serving as a rich source of information, BIOMATDB takes a step further in supporting companies, particularly SMEs, by facilitating market access for their biomaterial products. The Biomaterial Database is extended to include an information-oriented Biomaterial Marketplace, accessible via www.biomaterialmarketplace.com. This marketplace acts as a user-friendly and informative online web application instance, connecting suppliers and demanders in the biomaterial industry. Integrated Digital Advisors guide users through a step-by-step search process, streamlining interactions and transactions within the biomaterial market. This synergy between the Biomaterial Database and Marketplace accelerates the development and adoption of advanced bioinks, fostering collaboration and innovation in the field of 3D bioprinting.
Note: This article is based on Turunen, S. 2023. Biomateriaalista biomusteeksi. Solubiologi: Suomen solubiologit ry:n julkaisu, 41(1), pp. 20-26.
Author: Sanna Turunen
References
[1] Ozbolat, I. T., & Hospodiuk, M. (2016). Current advances and future perspectives in extrusion-based bioprinting. Biomaterials, 76, pp. 321-343.
[2] Murphy, S. V., & Atala, A. (2014). 3D bioprinting of tissues and organs. Nature Biotechnology, 32(8), pp. 773-785.
[3] Liu F., Quan R., Vyas C. & Aslan E. (2022). Hybrid biomanufacturing systems applied in tissue regeneration. International Journal of Bioprinting, 9(1), pp. 320-335.
[4] Liu F. & Wang X. (2020). Synthetic Polymers for Organ 3D Printing. Polymers, 12(8), 1765.
[5] Mancha Sánchez E., Gómez-Blanco J. C., López Nieto E., Casado J.G., Macías-García A., Díaz Díez M.A., Carrasco-Amador J.P., Torrejón M.D., Sánchez-Margallo F. M. & Pagador J. B. (2020). Hydrogels for Bioprinting: A Systematic Review of Hydrogels Synthesis, Bioprinting Parameters, and Bioprinted Structures Behavior Frontiers in Bioengineering and Biotechnology, 8, article 776.
[6] Turunen, S., Kaisto, S., Skovorodkin, I., Mironov, V., Kalpio, T., Vainio, S., Rak-Raszewska, A. (2018). 3D bioprinting of the kidney—hype or hope?, AIMS Cell and Tissue Engineering, 2(3), pp. 119-162.
[7] Masri S., Maarof M., Aziz I.A., Idrus R., Fauzi M.B. (2023) Performance of hybrid gelatin-PVA bioinks integrated with genipin through extrusion-based 3D bioprinting: An in vitro evaluation using human dermal fibroblasts. International Journal of Bioprinting, 9(3), pp. 422-442.
[8] Osidak E.O., Kozhukhov V.I., Osidak M.S., Domogatsky S.P. (2020) Collagen as Bioink for Bioprinting: A Comprehensive Review, International Journal of Bioprinting, 6(3), pp. 17-26.
[9] Jongprasitkul, H., Turunen, S., Parihar, V.S. & Kellomäki, M. (2023) Sequential Cross-linking of Gallic Acid-Functionalized GelMA-Based Bioinks with Enhanced Printability for Extrusion-Based 3D Bioprinting Biomacromolecules, 24(1), pp. 502-514.
[10] Jia, J., Richards D.J., Pollard, S., Tan, Y., Rodriguez, J., Visconti, R.P, Trusk, T.C., Yost, M.J., Yao, H., Markwald, R.R., Mei, Y. (2014) Engineering alginate as bioink for bioprinting, Acta Biomaterialia, 10(10), pp. 4323-4331.
[11] Ouyang, L., Highley, C.B., Rodell, C.B., Sun, W. & Burdick, J.A. (2016) 3D Printing of Shear-Thinning Hyaluronic Acid Hydrogels with Secondary Crosslinking, ACS Biomaterials Science & Engineering, 2(10), pp. 1743-1751.
[12] Naghieh, S. & Chen, X. (2021) Printability–A key issue in extrusion-based bioprinting, Journal of Pharmaceutical Analysis, 11(5), 2021, pp. 564-579.
[13] Schwab, A., Levato, R., D’Este, M., Piluso, S., Eglin, D. & Malda, J. (2020) Printability and Shape Fidelity of Bioinks in 3D Bioprinting, Chemical Reviews, 120(19), pp. 11028-11055.
Keywords
bioprinting, biomaterial, bioink, synthetic hydrogel, natural hydrogel, thermoplastics, printability